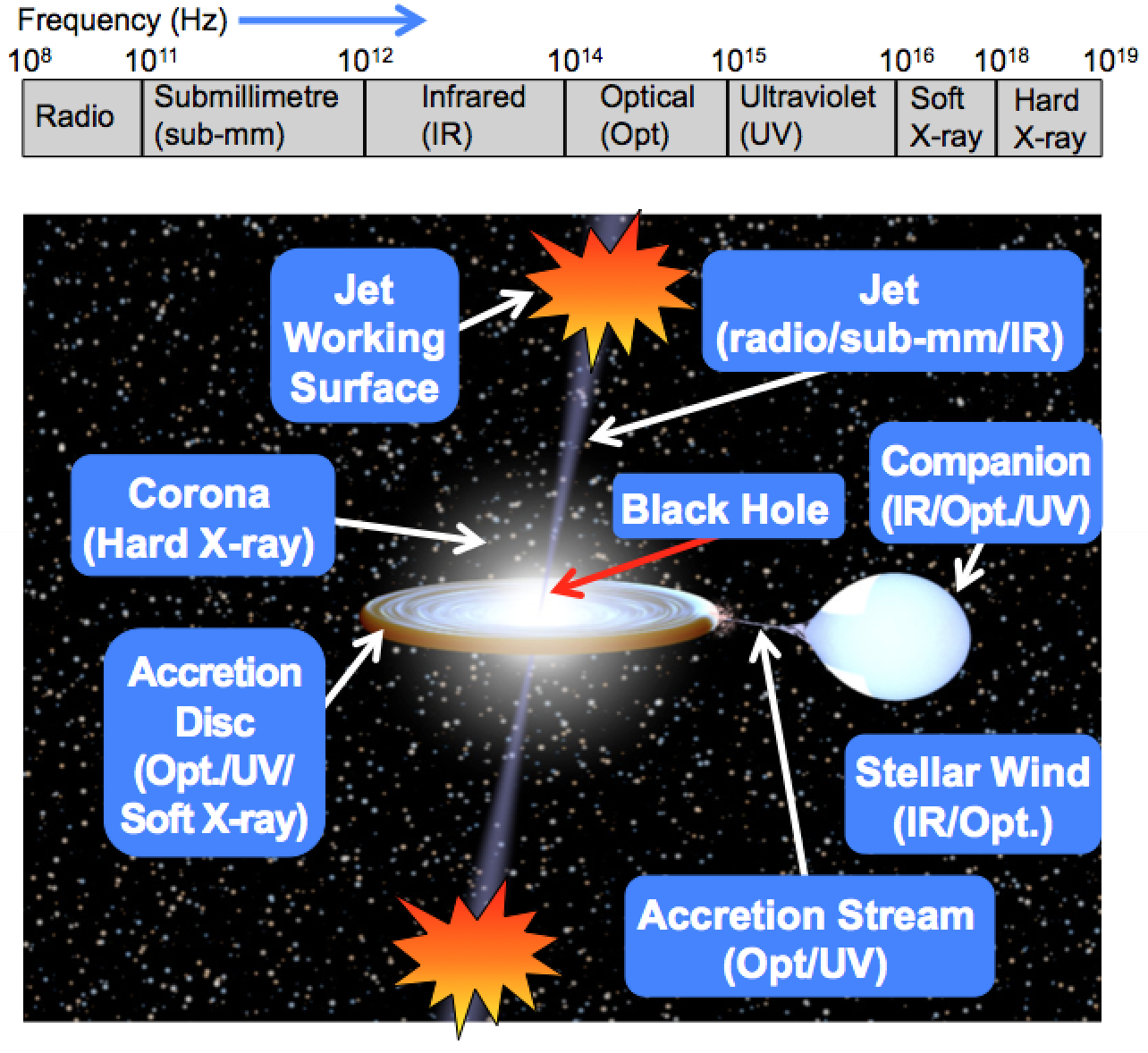
Spectral Domain
XB jets display a characteristic broad-band spectrum consisting of optically thick synchrotron emission extending from radio up to sub-mm or infrared frequencies, which breaks to optically thin synchrotron emission at higher frequencies (Fender, 2001, MNRAS, 322, 31; Corbel et al., 2002, ApJ, 573, L35). The spectral break is a key observational tracer of jet physics, as this portion of the spectrum probes emission from the most compact region of the jet, where particles are first accelerated up to high energies (Markoff, et al., 2005, ApJ, 635, 1203). The exact spectral shape (i.e., spectral index, location of the spectral break) depends on jet properties such as geometry, magnetic field structure, and particle density profiles, as well as the plasma conditions in the region where the jet is first accelerated. My team's recent work has revealed that the jet spectrum varies dramatically during an outburst (Russell et al., 2014, MNRAS, 439, 139; Russell et al., 2015, MNRAS, 450, 1745). We have shown that through identifying and tracking how the spectral break changes with time, we can track the evolution of the physical conditions in the jet acceleration region (e.g., radius, magnetic field). By coupling these changes to variations in the accretion flow, we aim to determine which accretion flow properties govern the launching and quenching of XB jets. Further, the scaling of the break with luminosity provides a key physical anchor needed to test and guide the development of relativistic jet simulations, ultimately allowing us to understand how the jet we observe is produced (Polko et al., 2014, MNRAS, 438, 559; Ceccobello, et al., 2018, MNRAS, 473, 4417). Obtaining data in the mm/sub-mm regime is crucial in tracking the spectral break (see Fig. 2), as these bands fill a 2 orders of magnitude gap in frequency in our broad-band coverage (Tetarenko, et al., 2015, ApJ, 805, 300; Tetarenko, et al., 2019, MNRAS, 482, 2950).
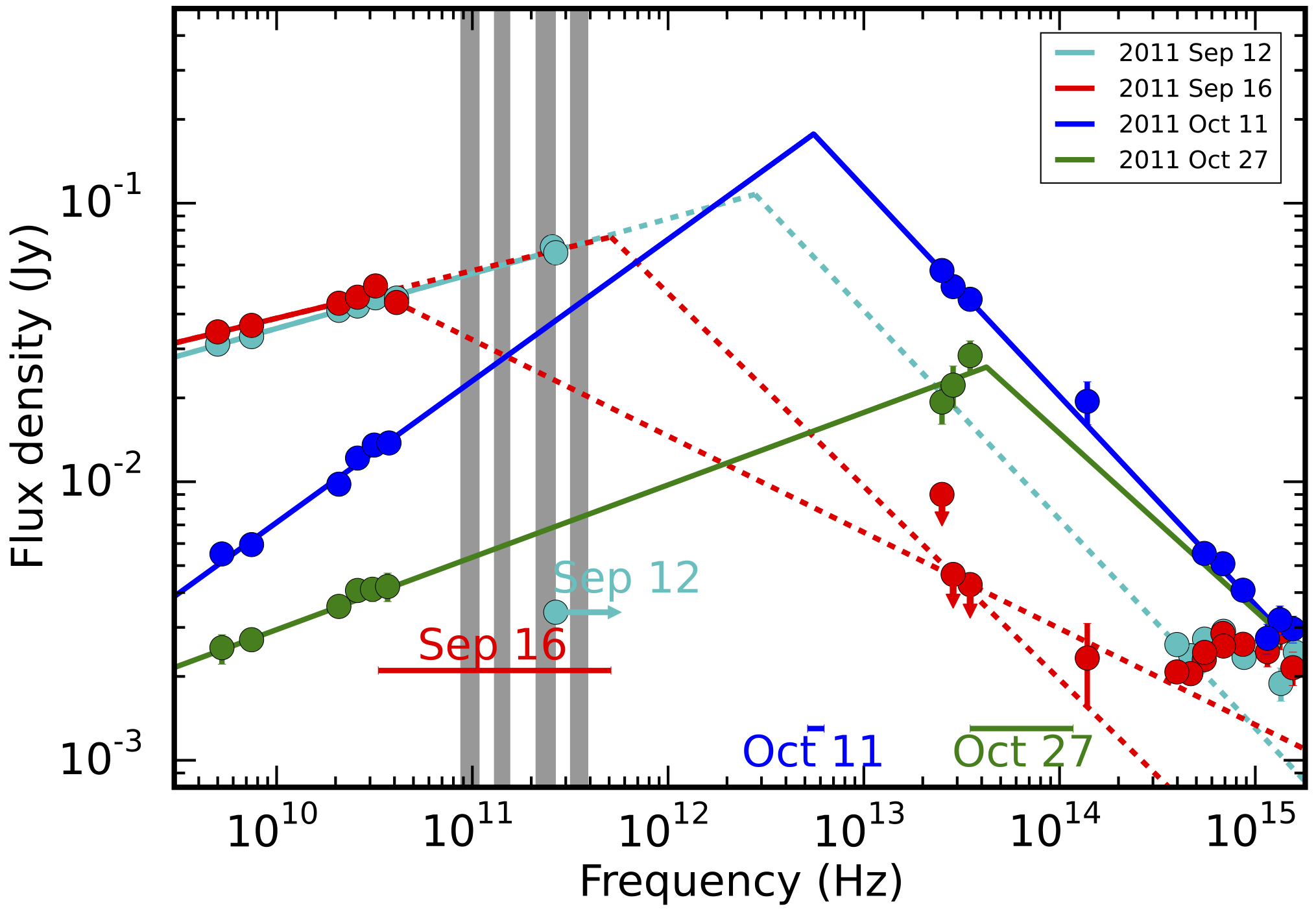
Temporal Domain
The emission observed from XBs can be strongly variable over a range of timescales (minutes to months). While broad-band spectral measurements and high resolution radio imaging studies with Very Long Baseline Interferometry (VLBI; e.g. Stirling et al., 2001, MNRAS, 327, 1273) are traditionally used to constrain jet properties, time domain observations offer a promising new way to address the key open questions in jet research (Uttley et al., 2015, SSRv, 83, 453). Detecting and characterizing rapid flux variability in jet emission from multiple BHXBs can allow us to probe detailed jet properties that are difficult, if not impossible, to measure by other means (e.g., jet geometry, speed, the sequence of events leading to jet launching; Casella et al., 2010, MNRAS, 404, L21; Gandhi et al., 2017, Nature Astronomy, 1, 859; Vincentelli et al., 2018, MNRAS, 447, 4524; Malzac et al., 2018, MNRAS, 480, 2054). While time-resolved observations are a staple for XB studies at higher frequencies (optical, X-ray; probing the accretion flow), there are many challenges that accompany such studies at low frequencies (radio, sub-mm; probing the jet). In particular, it can be difficult to disentangle intrinsic source variations from atmospheric variations, and the need to cycle between observing a target source and a calibrator prevents continuous data recording. Further, until recently, most telescopes were not sensitive enough, nor capable of taking the data to probe rapid (second) timescales. My team and I have been working on developing new observational techniques and computational tools (e.g., see CASA Variability Measurment Scripts) to overcome such limitations. This work allows us to accurately sample XB jets in the time domain at radio/sub-mm frequencies for the first time (see Fig. 3; Tetarenko et al., 2017, MNRAS, 469, 314), and connect jet variability properties with internal jet physics (Tetarenko et al., 2019, MNRAS, 484, 2987).
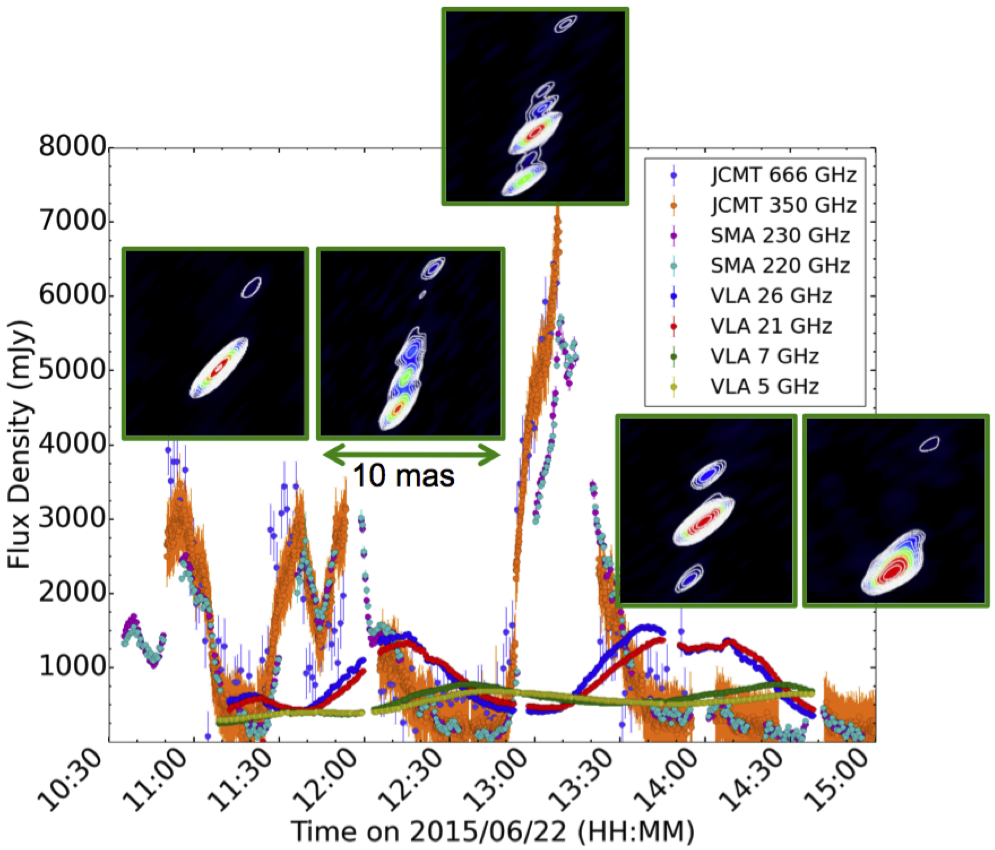
Jet-ISM Interactions
My team and I have developed a novel technique to identify and probe the regions where XB jets are colliding with the surrounding ISM (Tetarenko et al. 2018, MNRAS, 475, 448). Encoded within the physical conditions in these zones are valuable information on unknown jet properties, such as, the total jet power, jet composition, duty cycles, and the efficiency of jet feedback (Castor et al. 1975, ApJ, 200, L107; Heinz 2006, ApJ, 6363, 316). To take full advantage of these jet interaction regions to probe jet physics, we need to be able to efficiently identify these zones near multiple XBs. However, prior to our work there were only two BHXBs in our Galaxy with confirmed jet interaction sites (in the form of jet-blown cavities coincident with excited atomic/molecular gas) in the surrounding ISM (see Fig. 4; Gallo et al. 2005, Nature, 436, 819; Dubner et al. 1998, AJ, 116, 1842). Previous methods used to identify interaction zones near XBs involved a cumbersome multi-wavelength approach (radio + optical/X-ray), that hinges on detecting extended radio emission. This technique is expensive in terms of telescope time, and also very observationally difficult, due to the expected low surface brightness of such radio emission structures. Our new technique, using molecular line emission visible in the sub-mm bands to trace density, temperature, and the presence of jet driven shocks in these interaction zones, overcomes these complications; we can efficiently identify interaction sites, and accurately characterize the physical conditions of the ISM in these regions (needed to infer jet properties), with a short observation on a single telescope.
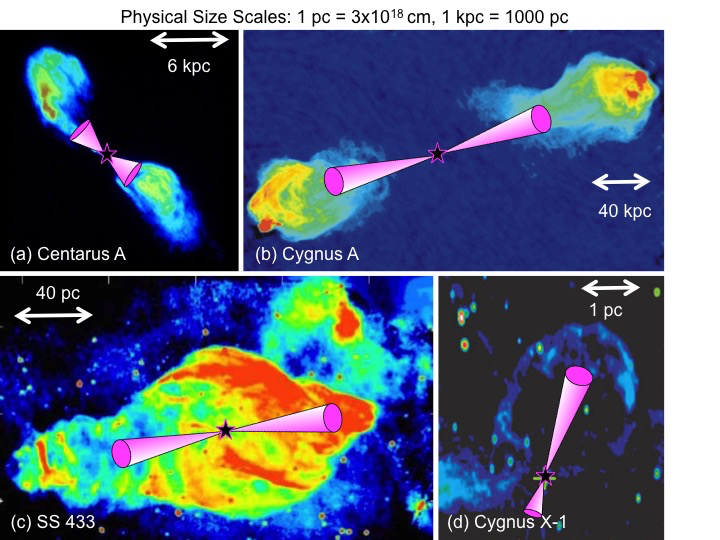
Disc-Jet Coupling
I have also done some work exploring the empirical disc-jet coupling relationship, relating radio and X-ray luminosity, in the XB population (see Fig. 5; Gallo et al. 2003, MNRAS, 344, 60; Corbel et al. 2013, MNRAS, 428, 2500). In particular, I focused on analyzing the mechanisms that govern radio luminosity in neutron star systems, where this coupling relationship had not previously been well studied. I worked to quantify the similarities and differences between this disc-jet coupling relationship in different accreting systems, to understand the key factors that may govern jet production and evolution (e.g., mass, spin, magnetic fields; Tetarenko et al. 2016, MNRAS, 460, 345; Tetarenko et al. 2018, ApJ, 854, 125).
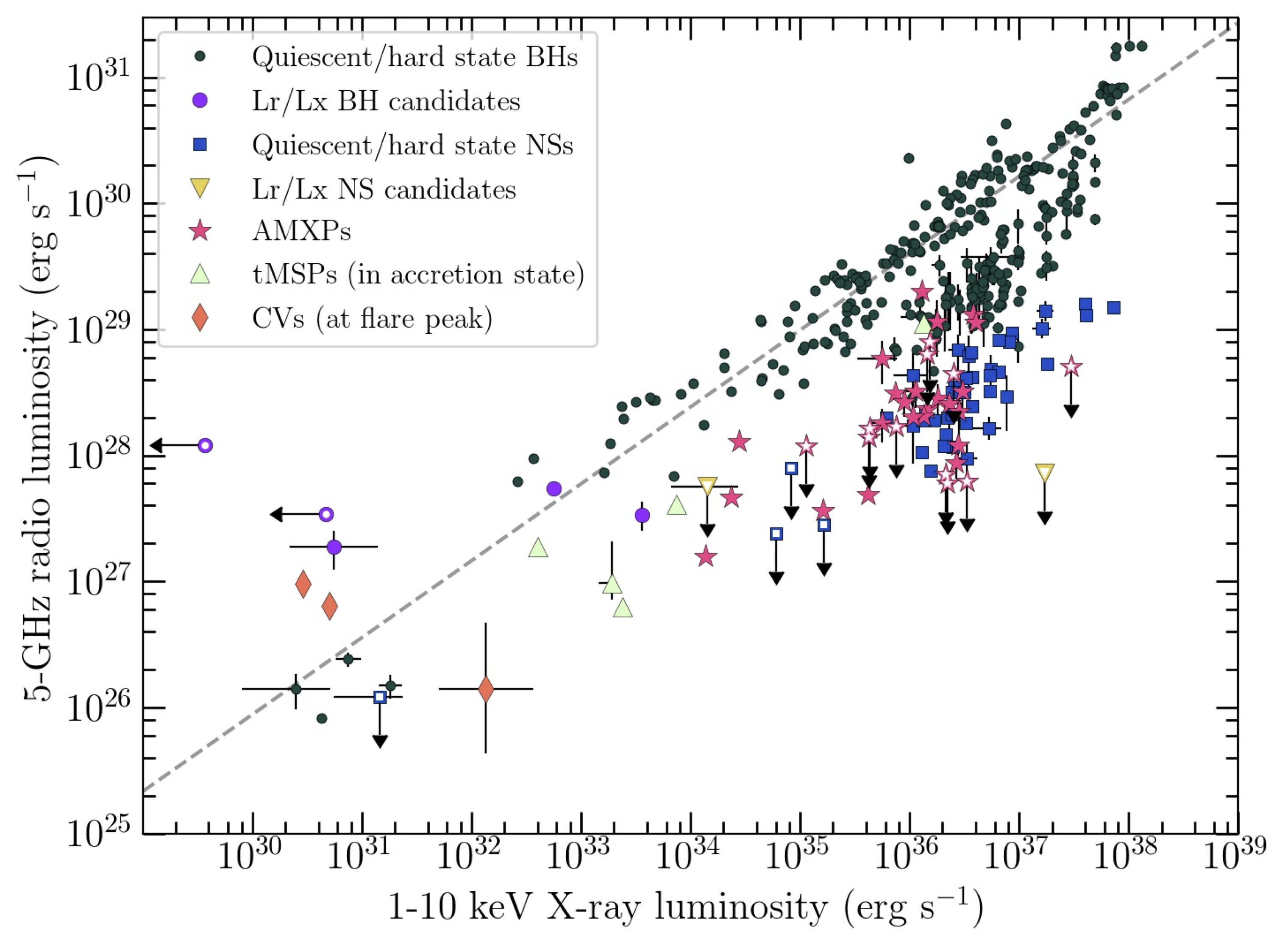
A large part of my work involves analyzing data from different telescopes around the world. The telescopes I rountinely use are shown in the right panel; the Karl G. Jansky Very Large Array (VLA), the Submillimeter Array (SMA), the James Clerk Maxwell Telescope (JCMT), the Northern Extended Millimeter Array (NOEMA), the IRAM 30m telescope, and the Atacama Large Millimetre Array (ALMA).